Exploring the Process of DNA to mRNA Translation
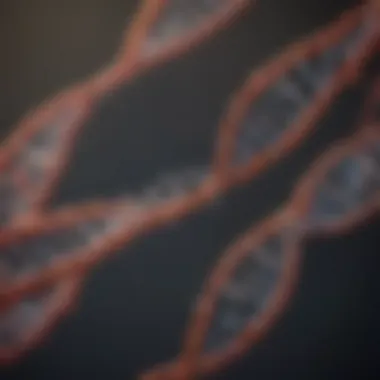
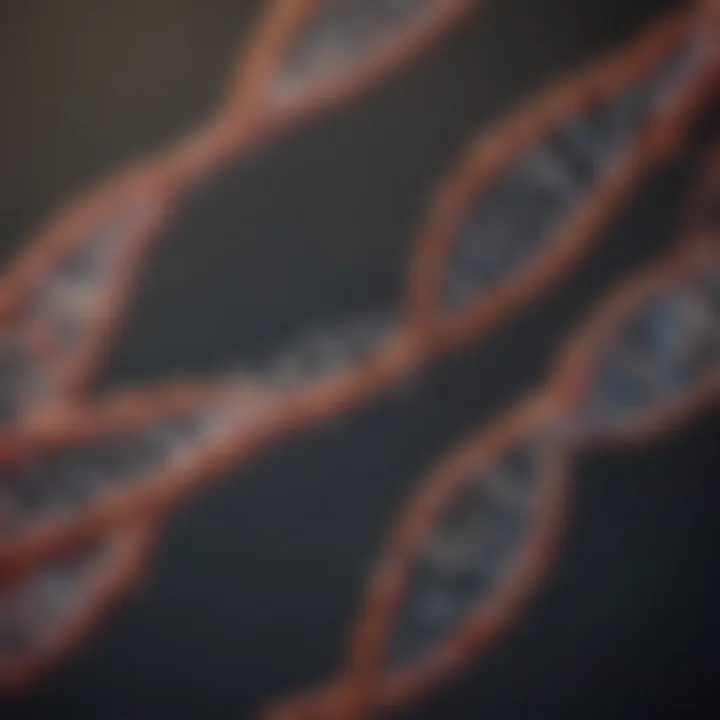
Intro
In the realm of molecular biology, one process stands out as a cornerstone of genetic expression: the translation of DNA into mRNA. This journey from the double helix of DNA to the single-stranded messenger RNA illustrates a captivating transformation that lays the foundation for protein synthesis. Understanding this process is not just an academic pursuit; it holds profound implications for the functioning of cells and the broader significance in biotechnology.
The process, often overlooked in its complexity, involves multiple key players, including RNA polymerases, transcription factors, and a makeup of regulatory elements. Each component has its distinct role in ensuring that the genetic instructions encoded within DNA are accurately transcribed into mRNA. What’s fascinating is how this translation affects cellular functions and how a deeper understanding could unlock new applications in genetic engineering and medicine.
Grasping the intricacies of how DNA transitions into mRNA provides valuable insights into genetic coding and expression. Whether you’re a student diving into genetics, a researcher pursuing advanced topics, or a professional in biotechnology, the details surrounding this transformation offer a wealth of knowledge. As we journey through this topic, we will dissect these components, their interactions, and the overarching implications they hold in various scientific discussions.
Prelims to Genetic Translation
The journey of translating DNA to mRNA is not merely a textbook occurrence; it's a vital mechanism that underscores the very essence of biological systems. This process is fundamental in converting the genetic information encoded within DNA into functional proteins, which perform countless roles in living organisms. Without translation, life as we know it would come to a standstill, making this topic indispensable for students, researchers, and professionals alike.
Understanding genetic translation is akin to decoding a complex language. Each part of the process contributes intricately to the synthesis of proteins that govern cellular functions, development, and overall homeostasis. It's essential to appreciate how these systems interact and evolve over time, as this knowledge not only deepens our comprehension of life at a molecular level but also paves the way for advancements in biotechnology and medicine.
By examining the translation process thoughtfully, we can uncover several key benefits:
- Novel Interventions: Insights into genetic translation can lead to groundbreaking gene therapies for various diseases, tailoring medical treatments to individual genetic profiles.
- Synthetic Biology: This area thrives on the manipulation of mRNA, creating new pathways for engineering organisms with desired traits or functions.
- Innovative Research: A solid grasp of translation underpins much of molecular biology, enabling researchers to explore genetic regulation and expression.
Defining the Genetic Code
The genetic code is universally revered as the blueprint of life. Well, at least that’s the popular sentiment. But what does it actually entail? First and foremost, it refers to a set of rules by which the sequence of nucleotides in DNA is translated into the amino acid sequence in proteins. It’s composed of codons, which are triplets of nucleotides that correspond to specific amino acids.
To clarify:
- Codon Composition: Each codon in the code translates to one of the 20 possible amino acids or signals when to start or stop protein synthesis.
- Universality: This code is nearly universal among all organisms, illustrating a common evolutionary origin, making it both fascinating and consistent across species.
- Redundancy: Interestingly, the genetic code is also redundant. Multiple codons can code for the same amino acid—take leucine, for example, which can be represented by UUA, UUG, CUU, CUC, CUA, and CUG.
Thus, while there might appear to be complexities in genetic translation, understanding the basics of the code serves as a springboard into deeper discussions about transcription and regulation.
The Importance of mRNA
Once we set aside the definition of the genetic code, we encounter one of its primary products: messenger RNA, commonly referred to as mRNA. This single strand of nucleotides acts as the bridge between DNA and protein. It carries the genetic instructions from the nucleus to the ribosomes, where proteins are synthesized.
The significance of mRNA cannot be overstated:
- Role as a Template: mRNA serves as a template that dictates the order in which amino acids are assembled into proteins, determining the structure and function of the resulting molecules.
- Regulatory Functions: Beyond mere information relay, mRNA plays a notable role in the regulation of gene expression, influencing how proteins are produced in response to various internal and external signals.
- Adaptation and Response: Cells can modulate the levels of different mRNAs according to their needs, enabling quick responses to environmental changes. This responsiveness is key for cell survival and adaptation.
"The mastery of mRNA's function is pivotal for maintaining life's intricate balance, being both a catalyst for change and a keeper of cellular homeostasis."
In summary, the translation from DNA to mRNA is not just a biological process; it's a foundational aspect that shapes everything from basic cell functions to sophisticated biotechnological applications. Understanding it opens the door to a multitude of scientific explorations, making the study of genetic translation one of the most compelling narratives in modern biology.
The Role of DNA in Protein Synthesis
DNA serves as the blueprint for life itself, guiding the intricate dance of protein synthesis. Without DNA, cells would find themselves without directives, akin to a ship lost at sea without a navigator. It holds the genetic instructions—the recipes—needed for the synthesis of proteins, which are crucial for structure, function, and regulation of the body’s tissues and organs. Delving into its role is not merely academic; it’s about understanding the fundamental principles of biology that dictate how living organisms operate.
Structure of DNA
The structure of DNA is a critical aspect of its function in protein synthesis. Two particulars shine prominently: double helix formation and nucleotide composition, both of which work together in a remarkable and efficient manner.
Double Helix Formation
The double helix formation of DNA is one of its most striking features. Picture a twisted ladder, where the rungs represent pairs of nucleotides and the sides are the sugar-phosphate backbone. This spiral structure not only provides stability but also makes replication a breeze. When it’s time for a cell to divide, each strand serves as a template to duplicate the genetic information.
One key characteristic of this formation is its ability to protect the bases within the helix from potential damage. The double helix keeps these essential components tucked away, enhancing genetic stability. Furthermore, this structure ensures that the base pairs align precisely, fostering accurate transcription into mRNA—a vital process for gene expression.
Despite its well-known advantages, the double helix also has disadvantages. For example, the tight winding can sometimes impede access for proteins that need to bind to DNA, creating challenges during transcription.
Nucleotide Composition
Moving on to nucleotide composition, this is where DNA really gets its specific character. Each nucleotide consists of a phosphate group, a sugar molecule, and a nitrogenous base. The order of these nucleotides stores all the genetic information, acting as a unique code for every organism.
The ability of nucleotides to form pairs is crucial. Adenine pairs with thymine, and cytosine pairs with guanine—this reliable pairing is vital for the accurate copying of DNA during replication. Moreover, the duos allow for the formation of numerous combinations, which ultimately leads to the vast diversity in genetic coding.
One unique feature of nucleotide composition is its dynamic nature; it can be influenced by various factors like environmental conditions or genetic mutations. While this flexibility enables adaptation, it can also lead to detrimental consequences, such as the emergence of genetic disorders or increased susceptibility to diseases.
Function of Genes
Genes, segments of DNA, perform specific functions that are integral to how proteins are synthesized, through processes that include gene regulation and expression patterns.
Gene Regulation
Gene regulation is akin to a conductor guiding an orchestra—ensuring that the right proteins are synthesized at the right time and in the right amounts. This control is vital for cellular functionality and adaptability. Without it, cells would act erratically, producing unnecessary proteins or failing to manufacture critical ones. Gene regulation involves various mechanisms, including enhancers and silencers that maximize or silence gene expression.
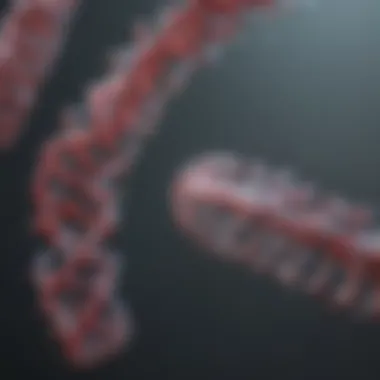
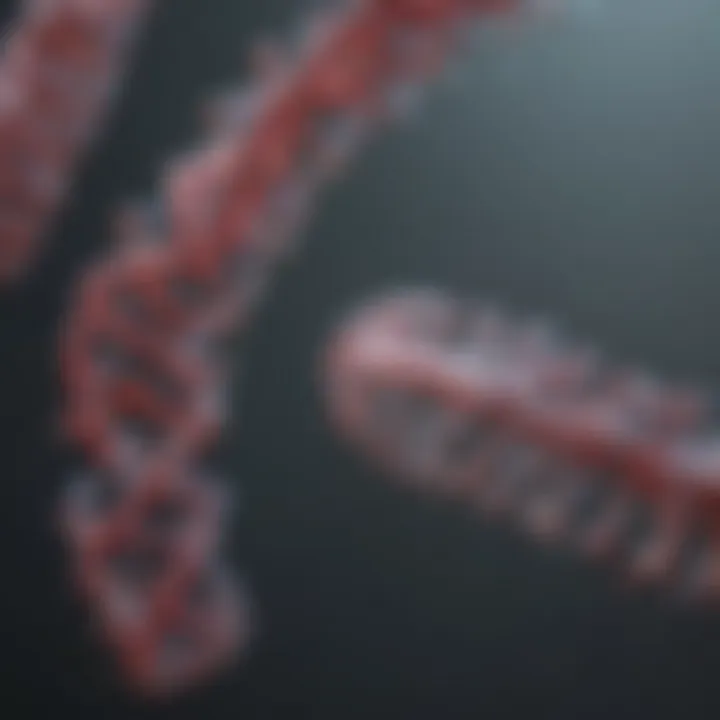
This characteristic of gene regulation also offers a beneficial aspect: it allows for cellular differentiation. Essentially, different cell types can arise from the same genetic material, contributing to the incredible diversity seen within multicellular organisms.
However, misregulation can lead to significant issues, such as cancer, where genes responsible for controlling cell division become dysfunctional.
Expression Patterns
Expression patterns are the distinct sequences that dictate how and when genes are turned on or off in a given tissue or time period. This aspect assumes great importance in the understanding of genetic expression. It factors in how all genes are not actively expressed in every cell, leading to the specialization needed for different tissues. For instance, muscle cells will express genes related to contraction that are not active in nerve cells.
The uniqueness of expression patterns adds another layer of complexity to molecular biology. This complexity can be beneficial by ensuring proteins are produced only when needed, but it can also complicate matters when trying to pinpoint how certain diseases develop based on misregulated expression.
"Understanding the role of DNA in protein synthesis is foundational to grasping the broader mechanisms of life at a molecular level."
Overall, the role of DNA in protein synthesis encapsulates several fundamental processes that govern life. The intricate relationship between structures, nucleotide composition, gene regulation, and expression patterns showcase just how sophisticated biological systems are. As research advances, the implications of these findings continue to expand, sparking interest across various fields, including medicine and biotechnology.
From DNA to mRNA: Translation Mechanics
The journey from DNA to mRNA is a crucial mechanism of life. It deserves a spotlight due to its central role in protein synthesis, determining the fate of cellular functions. This section zooms in on how the intricate steps of transcription underpin the entire translation process, setting the stage for translating genetic information into functional proteins. Understanding this mechanics not only aids in grasping biological processes but also shines light on potential implications in fields like medicine and biotechnology.
Transcription Overview
Transcription is the initial phase in the DNA to mRNA journey. It is the process through which the information encoded in a DNA sequence is converted into a complementary RNA strand. This overview comprises three distinct yet interlinked phases: initiation, elongation, and termination. Each phase plays its part in ensuring that the genetic blueprint is accurately read and replicated into mRNA, which will later be used in the translation phase of protein synthesis.
Initiation Phase
The initiation phase marks the first steps of transcription. A notable characteristic of this phase is the recognition of promoters. Promoters are specific regions on the DNA that signal where transcription should begin. RNA polymerase, the key enzyme in this process, binds to the promoter region, preparing to unwind the DNA strands.
One unique feature of the initiation phase is the formation of the transcription complex. This complex is a collection of proteins that assemble with RNA polymerase at the promoter. This phase is often regarded as critical as it sets up the framework for the entire transcription process. If the initiation does not occur efficiently, the translation of genes may falter later on.
Nevertheless, there are some potential pitfalls—such as mutations in promoter regions which can hinder proper transcription initiation. In summarized terms, a smooth initiation lays down the groundwork for successful transcription.
Elongation Phase
Once the initiation phase sets the wheels in motion, the elongation phase takes over, during which RNA polymerase moves along the DNA template strand, synthesizing mRNA. The primary characteristic here is that RNA strands are elongated as ribonucleotides are added, following the base pairing rules, though with uracil replacing thymine.
A unique aspect of the elongation phase is its speed. RNA polymerase can add ribonucleotides at a significant pace—tens of nucleotides can be added per second. This rapid synthesis ensures that mRNA is produced efficiently without unnecessary delays. While rapid synthesis is generally advantageous, mistakes can occur, resulting in errors that might lead to malfunctioning proteins—an aspect highlighting the trade-offs inherent to this phase.
Termination Phase
As transcription progresses towards completion, the termination phase kicks in. The hallmark of this phase is the sequence signals that indicate the end of transcription. At certain regions on the DNA, RNA polymerase recognizes these signals, which cues it to detach from the DNA and release the newly formed mRNA strand.
This phase is essential as it ensures that mRNA is accurately produced in its entirety, freeing it for the next stages of processing and ultimately, translation. However, incorrect termination can result in truncated or improperly formatted mRNA molecules, making this a vital checkpoint in gene expression.
Key Enzymes in Transcription
Transcription does not happen by magic; it relies on key enzymes, primarily RNA polymerases, that work tirelessly to create the mRNA template. Their functions can often dictate the efficiency and accuracy of the transcription process.
RNA Polymerase Functions
The central figure in this narrative is RNA polymerase, an enzyme with the primary function of synthesizing RNA from a DNA template. It possesses a remarkable ability to unwind the DNA helix and synthesize a new strand of RNA in the 5' to 3' direction.
A significant characteristic of RNA polymerase is its high fidelity; while it can make mistakes, it has proof-reading capabilities that allow it to correct errors as they happen. This feature is immensely beneficial for maintaining the structural integrity of the resulting mRNA.
Nevertheless, despite its beneficial traits, RNA polymerase is not immune to malfunctioning, which can lead to inaccurate transcription. Such discrepancies can have far-reaching consequences, emphasizing the necessity of understanding its functions.
Alternative RNA Polymerases
In addition to the main RNA polymerase (RNA polymerase II, mostly), there are also alternative RNA polymerases present in eukaryotic cells. These alternative RNA polymerases have specialized roles and contribute significantly to the transcription of different classes of RNA molecules. They showcase a crucial aspect of transcription's versatility.
The key characteristic of these enzymes is their specificity for different types of genetic sequences. For instance, RNA polymerase III primarily handles the synthesis of tRNA and small RNAs. This specialization speaks to the broader organizational needs of a cell.
While their specialized functions are advantageous, it can lead to complexities in regulation, as these different RNA polymerases must be precisely managed to ensure overall gene expression is harmonized. In short, both standard and alternative RNA polymerases are indispensable in the dance of transcription, each with their unique roles and challenges.
Regulatory Elements in Transcription
Transcription is a beautifully orchestrated process, but it does not happen in isolation. Instead, it is influenced by numerous regulatory elements that control the efficiency and timing of how genes are expressed. These elements serve as the gatekeepers of genetic information, ensuring that the right proteins are made at the right moment in a cell's life. Regulatory elements are crucial for precise gene expression and play a pivotal role in the overall functioning of organisms.
Promoters and Enhancers
Types of Promoters
Promoters are the initial landing pads where the transcription machinery assembles. They are typically found just upstream of the gene they control. There are several types of promoters, but the core promoter is the most significant. The core promoter, often characterized by a specific sequence termed the TATA box, facilitates the binding of RNA polymerase and necessary transcription factors. This makes it a key player for initiating the transcription process.
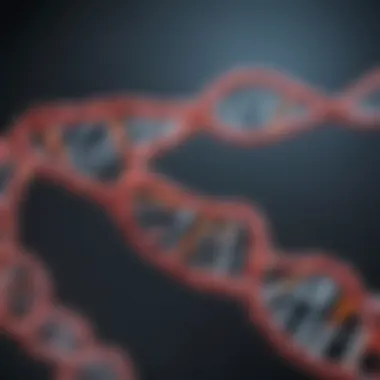
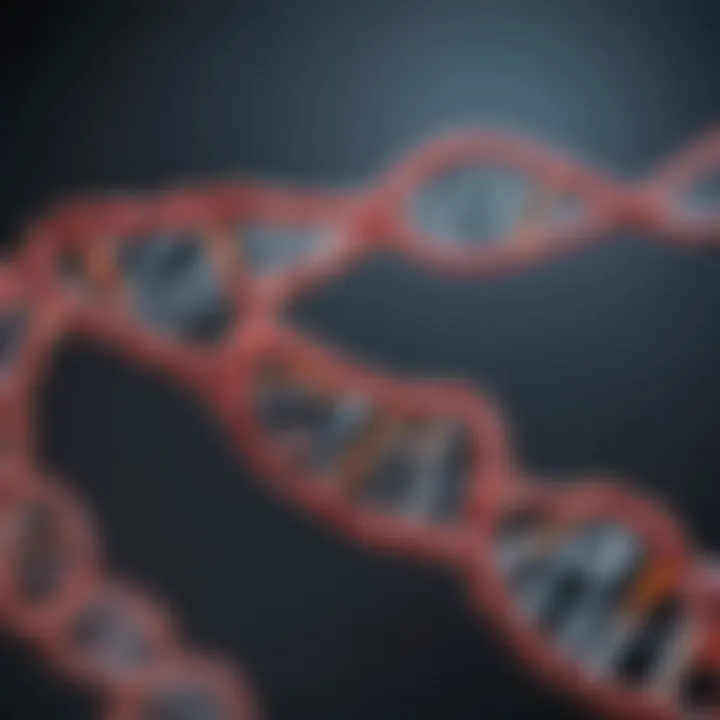
Promoters can be classified based on their strength, leading to an important consideration when discussing gene regulation. Strong promoters generate high levels of transcription, while weak promoters yield lower transcription rates. The unique feature here is their ability to control gene expression levels. Strong promoters are beneficial for genes that require consistently high expression, such as those that encode for ribosomal proteins, while weak promoters are often used for regulatory genes, where fine control is crucial.
Enhancer Mechanisms
Enhancers, on the other hand, are like distant cousins of promoters; they can exert influence over a gene's transcription from afar. They can be located thousands of base pairs away from the genes they regulate, yet they can loop back to interact with the transcription machinery at the promoter. The key characteristic of enhancers is their role in increasing the probability of transcription. They contain binding sites for transcription factors that, once attracted, help enhance the transcription level of specific genes.
A significant advantage of enhancers is their ability to be tissue-specific, meaning they can modulate gene expression in certain cell types but not in others. This feature is critical for processes like development, where different genes need to be expressed in different tissues. However, enhancers can also introduce complexities; mutations in enhancer regions can lead to misregulation of gene expression, which could cause diseases, reflecting a disadvantage that must be carefully navigated in genetic studies.
Role of Transcription Factors
Transcription factors are the unsung heroes that bring coordination to the transcription process. They bind to both promoters and enhancers, acting more like conductors of a symphony than mere assistants. These molecules dictate when, where, and how much of a gene product is made—a crucial aspect for maintaining cellular homeostasis and function.
General transcription factors
General transcription factors are necessary for the core transcription machinery to function. They assist in the assembly of the transcriptional complex, which is essential for DNA to be transcribed into mRNA. What makes general transcription factors a popular choice in discussions about gene regulation is their universal role across a wide variety of genes. They provide a baseline level of transcription, which is necessary for cellular function.
One unique feature is their ability to help stabilize the RNA polymerase binding at the promoter. While their presence is essential, their activity can also be modulated by specific transcription factors, showcasing how layers of control exist in gene expression.
Specific transcription factors
Diving deeper, we have specific transcription factors that can fine-tune the transcription process. Unlike general transcription factors, these are often cell or tissue-specific, meaning they can dramatically increase or decrease the transcription of specific genes in response to external stimuli. This specificity makes them vital in developmental processes and in the response to signals such as hormones or environmental changes.
The unique characteristic here is their adaptability; specific transcription factors can bind to multiple enhancer sequences and coordinate complex gene regulatory networks. However, this flexibility can also lead to competition for binding sites with other factors, potentially resulting in gene expression noise, a disadvantage that could throw a wrench into finely tuned cellular systems.
Processing of mRNA
The maturation of mRNA is a vital step in the larger process of gene expression, bridging the gap between DNA and protein synthesis. This segment focuses on how initial mRNA transcripts, known as pre-mRNA, undergo a series of modifications before they are ready for translation. Key adjustments such as capping and polyadenylation, as well as splicing, enhance the stability and functionality of mRNA, ensuring only the right sequences make it to the ribosome for protein crafting. The understanding of mRNA processing not only sheds light on fundamental biological processes, but also opens pathways for applications in gene therapy, synthetic biology, and biotechnology—fields that are rapidly evolving.
Capping and Polyadenylation
Capping refers to the addition of a modified guanine nucleotide to the 5' end of the mRNA molecule soon after transcription begins. This cap, often compared to a protective helmet, serves multiple purposes. First off, it guards the mRNA from degradation by exonucleases, enzymes that would otherwise break it down. Additionally, the cap plays a crucial role in ribosome recognition during translation initiation. It's like a VIP pass that ensures the mRNA is seen and used by the protein-synthesizing machinery within the cell.
On the other hand, polyadenylation occurs at the 3' end of the mRNA, where a long string of adenine nucleotides is added. This poly(A) tail not only promotes the stability of the mRNA but also aids in its export from the nucleus to the cytoplasm. The benefits of this modification cannot be overstated; a well-capped and polyadenylated mRNA is far less likely to be degraded and stands a better chance of being translated into protein.
A well-capped and polyadenylated mRNA is crucial for effective translation into proteins.
Splicing Mechanisms
Splicing is the process by which introns, the non-coding regions within a pre-mRNA transcript, are removed, and exons, the coding sequences, are joined together. This intricate dance of molecular machinery is carried out by a complex called the spliceosome, composed of several small nuclear RNAs and proteins. The precision of splicing is essential, as mis-splicing can lead to dysfunctional proteins that could cause diseases.
Moreover, splicing isn't just about cutting and pasting; it also allows for alternative splicing. This means that a single gene can produce multiple mRNA variants by including or excluding certain exons. This provides a layer of regulatory control and diversity in protein function, akin to how a musician can take a single melody and rearrange it in various ways to produce different songs.
The Role of mRNA in Protein Synthesis
mRNA, or messenger RNA, serves as the cornerstone of the protein synthesis process. By functioning as a template derived from DNA, it bridges the gap between genetic information and the resultant proteins that dictate cellular structure and function. This section spotlights two critical components of mRNA's roles: the translation process itself and the subsequent post-translational modifications that refine protein function.
mRNA Translation Process
Ribosome Assembly
Ribosome assembly is a crucial step in the translation phase of protein synthesis. The collaboration between mRNA and ribosomes lays the groundwork for synthesizing proteins. When translation kicks off, ribosomes come together around the mRNA strand, forming a functional unit capable of decoding the genetic instructions encoded within the mRNA.
One of the key characteristics of ribosome assembly is its ability to initiate quickly and efficiently. The ribosome works on a principle of fidelity, ensuring that the right tRNA molecules are paired with the mRNA codons. This precision makes ribosome assembly a popular choice when discussing translation processes.
A unique feature of ribosome assembly is that it is not a solitary process; instead, it is facilitated by numerous factors, including initiation factors, which assist in the proper assembly of the ribosome on the mRNA. While there are advantages to this intricate assembly process, such as high efficiency, a downside can include the complexity of regulation at various stages. Overall, ribosome assembly is fundamental to the accurate translation outcomes crucial for cellular function.
tRNA Functionality
As a player in the mRNA translation process, tRNA serves a pivotal role in the accuracy and efficiency of protein synthesis. Each tRNA molecule is specialized, with an anticodon that pairs with a corresponding codon on the mRNA, ensuring the correct incorporation of amino acids into the growing polypeptide chain.
A notable characteristic of tRNA functionality is its versatility. Each tRNA can be thought of as an adaptor, translating genetic code into functional proteins. This adaptability is a beneficial aspect, allowing cells to synthesize a vast array of proteins based on varied genetic instructions.
The unique feature of tRNA functionality lies in its distinct structure, which includes a three-leaf clover shape. This shape is vital for the interaction with both mRNA codons and amino acids. While tRNA's versatility and specificity are beneficial in producing a range of proteins, the potential disadvantage includes the need for the cell to maintain an array of tRNA molecules to ensure proper translation under different conditions, which can add to metabolic costs.
Post-Translational Modifications
Post-translational modifications are processes that further refine proteins after they are synthesized. These modifications ensure that proteins function correctly in various cellular contexts and extend beyond mere assembly into a vital stage of protein maturation.
Types of Modifications
Post-translational modifications encompass a range of chemical changes to proteins after translation, including phosphorylation, methylation, glycosylation, and ubiquitination. Each modification plays distinct roles, influencing protein stability, localization, and activity.
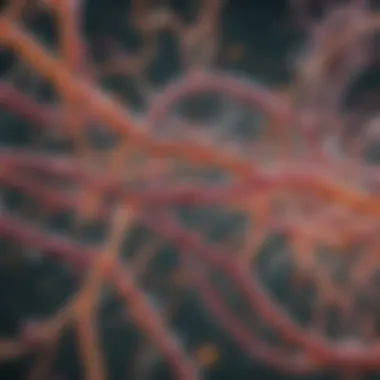
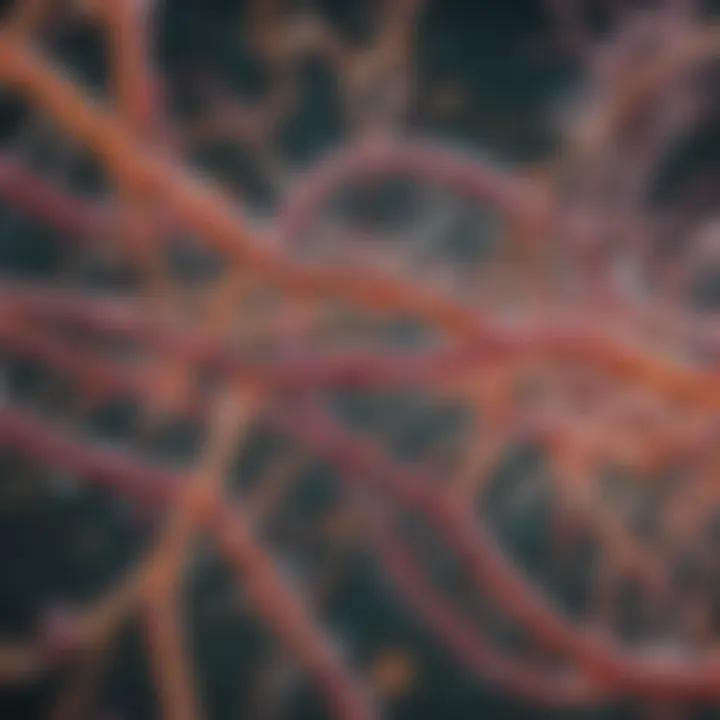
One key characteristic of types of modifications is their diversity. This diversity is beneficial because it allows proteins to achieve functional specificity in response to cellular signals or environmental changes. Unique features of these modifications include their potential reversibility, which enables dynamic regulation of protein functions. However, this complexity can pose challenges in terms of understanding and predicting protein behavior in different contexts.
Significance of Modifications
The significance of post-translational modifications cannot be understated, as these modifications can drastically alter a protein's life span, activity, and interactions. For instance, the addition of phosphate groups can activate or deactivate enzymes, affecting metabolic pathways.
A critical characteristic of the significance of modifications is their role in fine-tuning the cellular machinery. This regulation ensures that proteins operate within the right context at appropriate times, making it a valuable aspect of cellular control mechanisms. Unique features of these modifications include their impact on cellular signaling networks, which can have both beneficial effects, such as enhanced responsiveness to stimuli, and drawbacks, such as the potential for dysregulation leading to diseases.
Overall, the role of mRNA in protein synthesis highlights the intricate interplay between translation and post-translational processing—both essential for cellular function and life itself. Each step in this process adds layers of complexity, underscoring the importance of understanding molecular biology in a broader context.
Molecular Tools and Techniques
Molecular tools and techniques are the backbone of modern molecular biology research. They enable scientists to investigate the complex processes involved in the translation of DNA to mRNA. These methods facilitate the exploration of genetic material and provide essential insights into gene expression, regulation, and function. Without these tools, much of what we understand about genetic translation and its implications would remain shrouded in uncertainty.
In this section, we will delve into two crucial technologies: Polymerase Chain Reaction, commonly known as PCR, and various sequencing methods. Both play a significant role in biotechnology, driving advancements in healthcare, agriculture, and environmental science.
PCR and Its Applications
Polymerase Chain Reaction, or PCR, is a widely used technique that allows scientists to amplify specific segments of DNA. By turning a minuscule sample into a sufficient amount for analysis, PCR has transformed genetic studies.
- Amplification Process: The technique involves repeated cycles of denaturation, annealing, and extension. Each cycle doubles the amount of target DNA, exponentially increasing the sample size.
- Applications:
- Considerations: While PCR is exceptionally powerful, it's essential to maintain sterile conditions, as contamination can lead to incorrect results.
- Disease Diagnosis: PCR is crucial in identifying genetic disorders or pathogens. For example, it has been instrumental in diagnosing viral infections, such as COVID-19.
- Forensic Science: It can amplify DNA from crime scene evidence, enabling identification through DNA profiling.
- Cloning and Gene Expression Studies: Researchers use PCR to clone genes for further analysis or to express proteins in various systems.
Sequencing Methods
Sequencing methods determine the precise order of nucleotides in a DNA or RNA molecule. This information is vital for understanding genetic codes, variations, and function. There are several sequencing techniques, each with unique characteristics and applications.
- Sanger Sequencing: The first widely used method, allowing researchers to sequence small segments efficiently. It involves selective incorporation of chain-terminating inhibitors, ultimately revealing the DNA sequence.
- Next-Generation Sequencing (NGS): A revolutionary advancement that enables massive parallel sequencing. NGS has drastically reduced the time and cost of sequencing entire genomes.
- Third-Generation Sequencing: This method provides long-read technology, which is particularly useful for capturing complex genomes such as those of plants and certain animals.
- Applications: Genomic studies, personalized medicine, and the detection of genetic mutations.
"Molecular techniques like PCR and sequencing are not just tools; they are paving the way for discoveries that could change the world."
In summary, molecular tools and techniques serve as the essential instruments for dissecting the intricacies of genetic translation. They provide powerful avenues for exploration, enhancing our understanding of DNA and RNA mechanics, with far-reaching implications for many sectors, including health, agriculture, and beyond.
Implications in Biotechnology
The process of translating DNA into mRNA is not just a cornerstone of molecular biology; it serves as a catalyst for advancing biotechnology. Recent developments in this field hinge on the ability to manipulate these biological pathways to create therapeutic solutions and innovative technologies. The profound implications of mRNA translation extend across various domains, ranging from gene therapy to synthetic biology, each with its own nuances and potential outcomes.
This biological translation process lays the groundwork for technologies that can change the way we approach health and sustainability. Here's a closer look at specific elements that underlie its importance:
- Therapeutic Advances: By harnessing the power of mRNA, researchers have made significant headway in developing vaccines and treatments for various diseases. The rapid production of mRNA vaccines during outbreaks, like SARS-CoV-2, is a testament to the capability of this technology in addressing public health emergencies.
- Precision Medicine: mRNA is becoming a key player in tailoring treatments. Therapies can be designed to deliver specific proteins to replace deficient or malfunctioning ones, addressing genetic disorders at their root causes.
- Agricultural Enhancements: The principles of mRNA utilization are making waves in the agricultural sector too. Genetically modified organisms can be designed to express traits that improve yield and withstand pests, leading to innovations in food production.
Industry leaders and academic institutions are exploring the fine details of these approaches, considering factors such as specificity, efficacy, and ethical considerations surrounding their use.
Gene Therapy Approaches
Gene therapy is among the most promising applications of the DNA to mRNA translation process. It revolves around correctly introducing genes into a patient's cells to treat or prevent disease. The delivery methods often use modified viruses as vectors to transport the desired mRNA effectively into the target cells.
- Mechanism: An effective gene therapy approach usually involves the delivery of a corrected gene that compensates for the faulty one. Once inside, the cell machinery translates this mRNA into functional proteins, ideally leading to restored normal function.
- Challenges: However, this avenue is not without its challenges. Factors like immune response, delivery efficiency, and gene expression levels are pivotal in ensuring successful outcomes.
The hope is that further refinement of these therapies could lead to breakthroughs in treating genetic disorders like Cystic Fibrosis and Hemophilia.
Synthetic Biology Developments
Synthetic biology is carving out a promising track in biotechnology, intertwining with the mRNA translation process to create new biological systems and organisms. This approach combines principles from biology and engineering to design and construct new biological parts, devices, and systems.
- Innovative Constructs: Synthetic biology facilitates the design of novel organisms that can produce mRNA to yield proteins of interest, potentially leading to the production of pharmaceuticals or biofuels in a more efficient manner than traditional methods.
- Future Prospects: Researchers are also exploring how to create synthetic gene networks that mimic or enhance natural processes, paving the way for future advancements in metabolic engineering and ecological resilience.
"Synthetic biology is redefining what's possible in biotechnology by allowing us to engineer life itself, providing tailored solutions to some of the world's most pressing challenges."
The End and Future Perspectives
The DNA to mRNA translation process is not just a key player in the orchestra of cellular function, but it also serves as the groundwork for understanding genetic expression and the nuances of biotechnology. This article has explored the numerous intricate steps involved in this translation, emphasizing how essential molecules like RNA polymerase and transcription factors orchestrate gene expression with finesse.
In summarizing our findings, it’s clear that the translation process is multifaceted. For instance, the role of regulatory elements can’t be overstated; these components ensure that genes are expressed when and where they are needed, acting like the conductors guiding an ensemble. Understanding this computationally complex arrangement helps researchers devise innovative gene therapies and synthetic biological systems that could reshape medicine and industry.
Summary of Findings
- The Mechanisms of Transcription: We dissected how transcription begins with the initiation phase, continuing through elongation, and culminating in termination. Each of these steps is crucial for producing an accurate mRNA strand, which is the blueprint for protein synthesis.
- Key Players: RNA polymerase stands out for its central role in synthesizing mRNA, while transcription factors ensure that specific genes are turned on or off depending on cellular needs. Their balanced interplay points towards a regulatory architecture that is remarkably dynamic.
- Processing of mRNA: After transcription, mRNA undergoes crucial modifications like capping and splicing. These ensure the mRNA's stability and readiness for translation, underscoring that the fate of an mRNA molecule isn’t merely determined by its sequence but also by how it is managed post-synthesis.
"Transcription is not a simple photocopying process; it’s an adaptive and intricate reimagining of genetic instructions."
Future Research Directions
- Exploring Alternative Splicing: Delving deeper into alternative splicing mechanisms holds promise for unraveling the complexities behind different protein isoforms. Understanding this could expand our knowledge of protein diversity and function.
- Biotechnology Applications: There's a silent revolution happening in synthetic biology where engineered transcription factors could usher in new avenues for gene editing. Research into how we might manipulate these factors offers enticing prospects for personalized medicine.
- Impact of Environmental Factors: Investigating how external stressors affect transcription regulatory mechanisms will yield insights into cellular responses to the environment. Examining this relationship can also highlight significant implications for understanding disease mechanisms and evolutionary adaptations.
- mRNA Therapeutics: The rise of mRNA vaccines during the pandemic has opened new doors for therapeutic applications. Further exploration into mRNA delivery systems and stability could future-proof these technologies against evolving pathogens.
By understanding each aspect of the DNA to mRNA translation process comprehensively, we can pave the way for innovative research and applications that will benefit human health and society as a whole.